Nuclear structure
How does the structure of the atomic nucleus arise out of the interactions between its constituent nucleons, i.e., the protons and neutrons? This is the fundamental question of ab initio nuclear theory. Placing our understanding of nuclei on a fully predictive footing, starting from the nucleon-nucleon interactions, is a key link in our first principles description of the physical world. Given recent advances in deriving nucleon-nucleon interactions from the quarks and gluons of quantum chromodynamics, ab initio nuclear theory bridges the gap between the fundamental Lagrangian field theories of nature and the properties of ordinary matter, as well as how the matter around us came to be, through reactions over the astrophysical history of the universe.
Our interest is in obtaining an understanding of the complex, highly-correlated structure of the nucleus as a quantum many-body system of interacting nucleons. We seek to understand how, out of this complexity, simplicity again arises, in the form of emergent collective degrees of freedom. Collective phenomena include rotation, nuclear deformation, nuclear superfluidity, and the condensation of tightly-bound clusters of nucleons into molecule-like structures within the nucleus.
The nuclear many-body problem is too complex to be addressed by paper-and-pencil methods alone. Even with the most advanced supercomputing, it remains massively computationally challenging. Our approaches to the problem combine state-of-the-art supercomputing, theoretical many-body physics, applied mathematics, and the mathematics of symmetries (group theory).
Continuing into heavier nuclear mass regions, we explore the properties of nuclei at the limits of angular momentum, proton/neutron ratios, and mass. Exotic forms of nuclear excitations, such as wobbling, chirality, and magnetic rotation, are investigated. Our fundamental nuclear structure calculations are used to provide important nuclear input properties for simulations of astrophysical environments (see Nuclear astrophysics below). We calculate level densities and electromagnetic strength functions for unstable nuclei far from the realm of normal nuclei. Our calculations serve as testbeds for experimental studies of unstable nuclei at facilities including Notre Dame's own Nuclear Science Laboratory and the Facility for Rare Isotope Beams (FRIB).
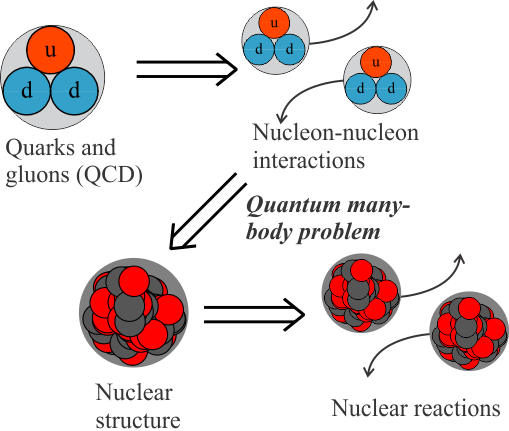
Nuclear astrophysics
Although many properties of nuclei can be explored in the laboratory, there are some that can only arise in explosive high temperature and high-density environments of stars and the big bang. In the nuclear astrophysics group at Notre Dame, the roles of fundamental nuclear properties and their interactions are studied in a host of astrophysical environments including the big bang, exploding supernovae, interiors of stars, collapsing black holes, energetic jets, and the dynamics of galaxy formation and evolution.
In an exploding supernova, one must understand nuclei in a range of temperatures up to 10 billion degrees and densities as high as a billion tons compressed to the size of a sugar cube. In such environments strange new phases of nuclear matter occur. These involve such exotic matter as "nuclear pasta" and quark-gluon plasma. We make detailed studies of how such new phases of matter affect supernovae and how we might detect their presence either in the neutrinos emanating from supernovae, black hole formation, or the properties of remnant neutron stars.
We also explore the detailed nuclear reactions responsible for the synthesis of the elements in nature via stars and stellar explosions. These studies clarify how the elements have arisen in nature and pin down the environments of the stellar furnaces from which they were forged. One particular focus is the mysterious origins of the heaviest elements such as platinum and uranium. We examine potential astrophysical sites of their synthesis, which include supernovae, magnetic jets from collapsing stars and black holes, and compact object mergers, and make explicit links between nucleosynthesis simulations, spectroscopic observations of old stars, and nuclear properties of extremely neutron-rich nuclei.
Finally, the big bang itself undergoes a rapid series of nuclear reactions leading to the formation of the light elements - hydrogen, helium, lithium - as the universe cools to a temperature of about 100 billion degrees. The details of this nuclear reaction epoch are sensitive to aspects of the origin and evolution of the universe itself from the first moment of creation of space-time through a variety of exotic phase transitions. We study how details of the process of big bang nucleosynthesis can constrain possible scenarios for the birth of the universe.
Nuclear theory research at Notre Dame is supported in part by the U.S. Department of Energy, Office of Science, Office of Nuclear Physics, under Award Numbers DE-FG02-95ER-40934 and DE-SC0013039, and the National Science Foundation under Grant No. PHY-1430152 (JINA Center for the Evolution of the Elements)
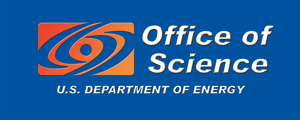
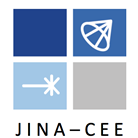